Sections
Evolutionary principles are the key to engineering enzymes for industrial applications.
Enzymes are natural catalysts that operate in every living cell, making life possible. Engineers have taken advantage of the catalytic properties of certain useful enzymes by removing them from their cellular environment and applying them in industrial applications. Enzymes can be used to create new products and simplify synthetic pathways. Today, they are the first choice for synthesis of chiral alcohols and amines in the pharmaceutical industry and complex oligosaccharides for the food ingredient market.
Laboratory breakthroughs in the application of evolutionary principles have led to a boom in new enzyme applications. Enzymes can now be trained as catalyst “athletes” capable of very high efficiencies. Once the right enzyme is identified for a process, enzymes are simple to apply — and they can reduce waste and streamline chemical processes.
What is special about enzymes?
Catalysts make chemical reactions easier, steering the chemical conversion to the desired product with less energy input than would be required without a catalyst. In addition to the arsenal of traditional chemical catalysts, ranging from acid/base to transition metal compounds, nature provides catalysts that obey the same chemical principles. In cells, the vast majority of natural catalysts are constructed from chains of amino acids called enzymes.
Within their well-structured polypeptide chains, enzymes have an active site that binds the substrate. Once the substrate is bound, the enzyme reduces the energy of the transition state of the reaction. The product is formed and then released from the active site (Figure 1).
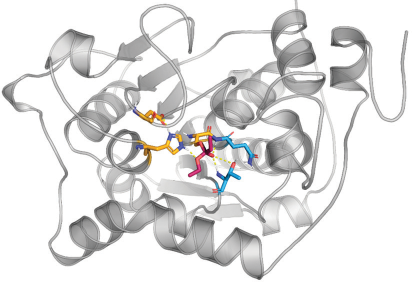
▲Figure 1. Lipase, a high-performance enzyme (gray), has bound the substrate (red) at the active site. Three well-positioned amino acid side chains (yellow) carry out the catalysis. Other amino acids (blue) position the substrate and reduce the energy of the transition state. The bulk of the polypeptide chain around the active site — i.e., the spirals, arrows, and loops — define the characteristics of the catalyst.
Only a few amino acids within a polypeptide chain of several hundred amino acids carry out the actual catalysis. The rest of the amino acids are involved in:
- precise positioning of the active site
- building a pathway for the substrate to enter and product to exit
- defining the solubility properties of the enzyme particle
- adding areas of further control (e.g., inhibition and activation by binding other compounds)
- enabling the enzyme to pulsate to support catalysis.
All of these aspects define the characteristics of the enzyme, which makes them very complex catalysts (1).
Using enzymes in chemical processes
Enzymes can be applied in chemical processes — after all, they are just catalysts that behave according to chemical principles. Of course, they have special handling needs and specific application windows, just as traditional catalysts like Raney nickel or iron oxide do. Much like homogenous catalysts, enzymes require an aqueous environment and a stable pH. Application of homogeneous enzymes in an industrial setting requires little more than a stirred-tank reactor, a temperature range of 30–70°C, and a specific pH range.
For many processes, such as synthesis of organic compounds that are not easily soluble in water, organic cosolvents like isopropanol or dimethyl sulfoxide (DMSO) need to be added. Although this can be generally harmful to enzymes, some enzymes are remarkably stable in the presence of organic solvents. Certain enzymes can even withstand up to 50% isopropanol. Enzymes that are not compatible with solvents can be engineered to survive these conditions.
Although enzymes are soluble in water, they can also be attached to a substrate or support to form a heterogenous catalyst. The solid support is typically a granular material, such as diatomaceous earth, silica, or synthetic polymers. The immobilized enzyme can then be used in a packed bed reactor. Heterogenous catalysts are often reused to reduce costs.
A critical factor when using enzymes is the temperature of the process. General kitchen knowledge teaches us that proteins denature when they are heated above ~55°C, thereby losing their structure and function — turning egg white from clear to white and meat from raw to edible. This is true for the majority of proteins, and it has created the impression that proteins are very sensitive and not compatible with the harsh conditions of a chemical reactor (2). However, certain enzymes isolated from microorganisms are highly thermostable and capable of surviving in extreme environments, like hot springs and salt lakes.
In starch liquefaction processes, for example, wild-type enzymes are used in chemical reactors at temperatures above 55°C. Amylases and additional hydrolytic enzymes convert starch into glucose, which is further processed by the enzyme glucose isomerase to high-fructose corn syrup (HFCS). This process is conducted above 60°C to lower viscosity, inhibit microbial growth, and increase the reaction rate.
The starch liquefaction process also illustrates the benefits of applying enzymes to make new products. The chemical conversion of glucose to fructose has been known for more than 100 years. It requires a high pH and temperature, and typically generates a product with a low fructose concentration and side products, making it unsuitable for commercial food applications. However, the enzymatic process takes place at neutral pH and moderate process temperatures, and it produces HFCS with a high fructose content and a pleasant taste profile. Immobilization of the enzyme lowers its cost, making the process commercially viable.
For HFCS production, the wild-type enzymes perform well. However, wild-type enzymes that are specialized in their natural environment are usually not adequate for industrial applications. This is where evolution comes into play.
Let evolution take over
Enzymes have evolved to catalyze thousands of different reactions and perform adequately under the conditions of the cellular environment. Evolution is a simple and extremely powerful algorithm of mutation and selection that is responsible for diversification, adaptation, optimization, and innovation in the living world (3). Over millennia, evolution has imbued enzymes with their different functionalities and characteristics.
Given evolution’s ability to create such a diverse, powerful, and specialized arsenal of enzymes, engineers are harnessing evolution to optimize enzymes for use in chemical plants. High-performance athletes need training, and the training schedule for enzymes is the evolutionary algorithm. The evolutionary principles of natural selection — variation, inheritance, and selection — have spread rapidly into academic developments and industrial applications, which is the best proof of their success.
Starting in the 1960s, scientists tried to shortcut evolution through analysis of the enzyme structure, assuming that they could predict which amino acids needed to be changed to adapt enzyme function. Biochemists began to elucidate enzyme structures by making changes in their DNA that introduced targeted amino acid exchanges into the encoded enzyme. From this work, however, they learned that enzymes are not that easy to understand, and the bulk of the polypeptide chain around the active site plays a role in enzyme function. Even today, predicting the influence of mutations within enzymes is nearly impossible due to their complexity. However, computer and bioinformatics analyses are taking on an increasingly important role in the analysis of data and the generation of new hypotheses.
The breakthrough in enzyme engineering came in the early 1990s when the evolutionary algorithm was transferred to the lab (Figure 2). This process, known as directed evolution, involves the random mutagenesis of enzymes, rather than intelligent examination of the enzyme and creation of rational mutants. Random mutagenesis was key to helping scientists identify beneficial mutations, but the scientific principles behind many of the mutations are difficult for scientists to explain. Directed evolution is very powerful for optimizing enzymes for a desired reaction. Instead of predicting mutations, enzyme engineers determine how many mutations need to be added, how many variants need to be screened, how to create libraries, etc.
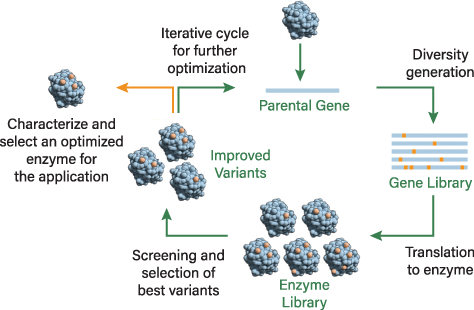
▲Figure 2. This diagram illustrates the design algorithm for enzyme evolution. The introduction of mutations takes place at the DNA level, producing a library of genes that in turn encode for a library of enzymes. Scientists screen for a set of enzymes with improved properties for the desired application. Variants can enter the cycle again for further optimization.
The first law of directed evolution was formulated by Nobel laureate Frances Arnold: “You get what you screen for.” Accordingly, the ultimate challenge of directed evolution is to implement screening systems to provide meaningful measures of the thousands of enzyme variants. The first screening systems used chromogenic substrates to monitor enzyme activity, which provided a visual or photometric readout. These systems provided quick access to enzymes with improved properties. However, the enzymes were improved only for the particular chromogenic substrate. The lab findings often did not translate into an effect on the desired reaction, which did not necessarily contain chromophores.
Several other screening systems have been investigated, including growth selection, agar-plate screens, and coupled reactions that detect side products. These methods suffer from either low accuracy, high complexity, or are too specific for a particular reaction. The current preferred method for industrial enzyme development is high-performance liquid chromatography (HPLC) analysis, which enables desired reactions to be monitored in a microtiter plate screening format (Figure 3). This method is broadly applicable to a range of reactions. However, the challenge is to replicate the process conditions of the desired reaction in an accurate microtiter plate-compatible analytical system.
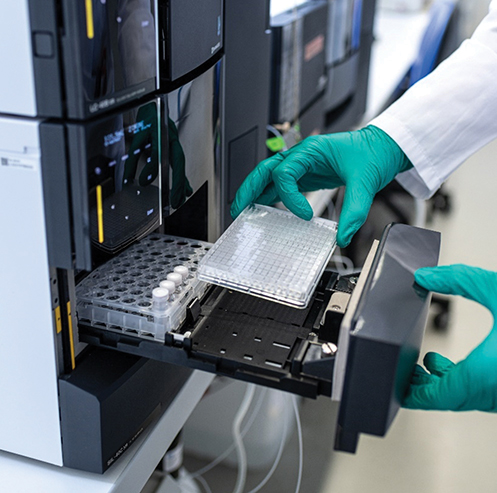
▲Figure 3. An engineer from c-LEcta prepares an enzyme reaction in a 384-well microtiter plate for high-performance liquid chromatography (HPLC) analysis, which has become a standard tool for industrial enzyme development.
Examples of real high-performance enzymes
In this section, a few examples illustrate the wide range of enzyme applications across various sectors, as well as the capabilities of enzyme engineering to optimize certain enzyme parameters (Table 1).
Table 1. Selected examples of engineered enzymes demonstrate the broad applicability of enzymes and the potential of enzyme engineering to enable new applications. | |||
Name | Function | Application | Properties Improved by Engineering |
Subtilisin | Protein hydrolysis | Detergent additive | Stability in detergents, oxidative stability, pH profile, substrate specificity |
Phytase | Hydrolysis of phytate, phosphate liberation | Animal feed additive | Stability in pelleting process, activity in the digestive tract of the animal (37°C and acidic pH) |
Transaminase/Alcohol dehydrogenase | Synthesis of chiral amines and alcohols | Active pharmaceutical ingredient (API) synthesis | Substrate scope, stereoselectivity, process stability, activity |
Sugar transferases | Synthesis of complex oligosaccharides | Food ingredient synthesis | Selectivity, process stability, increase in synthesis/hydrolysis ratio |
Detergent additives. A very early example of the use of enzyme engineering for an industrial application is the protease subtilisin used in detergents. The subtilisin enzyme was engineered to survive in the alkaline conditions of detergents and perform well at different washing temperatures. Subtilisin was comprehensively engineered and characterized to produce a large portfolio of subtilisin variants that suit the different, and ever changing, detergent formulation needs. Enzyme companies can respond quickly to changes in customer demands by providing optimized enzymes from this portfolio.
Animal feed additives. Phytase is an enzyme added to feed that hydrolyzes phytic acid to release phosphate. Its effect on animal nutrition is to increase the bioavailability of the phytate-bound phosphorous and diminish the antinutritional effect of phytate, which improves calcium, zinc, and iron utilization in animals. Although wild-type enzymes catalyze the reaction adequately, the requirements for the enzyme in this application are quite demanding (for more details, see Chapter 3.3 in Ref. 1). The enzyme needs to effectively hydrolyze phytate-bound phosphorus in the upper digestive tract of the animal. The body temperature of the animals is about 37°C and the environment is acidic, which is typically outside the comfort zone of the enzymes. Moreover, the enzyme must withstand the feed pelleting process, which occurs at temperatures of 65–80°C. Phytase was engineered to withstand these complex requirements, making it the dominant feed enzyme on the global market.
Active pharmaceutical ingredients (APIs). In the previous examples, enzymes are used to degrade molecules. The use of enzymes to instead synthesize molecules is a growing application field in the pharmaceutical and chemical industries. Enzymes are currently the first choice for the synthesis of APIs, such as chiral alcohols and amines. Enzymes are intrinsically stereoselective, but cannot necessarily be used to produce synthetic molecules that do not exist in nature. Enzyme engineers can manipulate enzymes to synthesize a broad range of chiral alcohol and amines that cannot be produced by wild-type enzymes. In many cases, enzymes achieve better performance than classical catalysts for these molecules.
An impressive example of enzymatic chiral amine synthesis is the process developed by Merck and Codexis to manufacture sitagliptin, a leading drug for the treatment of Type 2 diabetes. They replaced a chemical process using a Rh-t-Bu-Josiphos catalyst for asymmetric hydrogenation with an enzymatic pathway. However, the wild-type enzyme the researchers intended to use did not synthesize sitagliptin. Preliminary enzyme engineering produced a first variant with very low activity, i.e., 0.2% conversion of 2 g/L substrate using 10 g/L enzyme. After several rounds of directed evolution, the final enzyme variant was able to convert 200 g/L ketone to sitagliptin with excellent selectivity (99.95% enantiomeric excess [ee]) at 92% yield using 6 g/L enzyme in 50% DMSO (4). The new, streamlined process eliminates the need for high-pressure hydrogenation, the transition metal catalyst, and the chiral purification step. The enzymatic process increases the overall yield of the synthesis process by 13% and reduces waste generation by 19%.
Food ingredients. Linking sugar compounds can be a nightmare for synthetic chemists. The many linkage opportunities require stepwise protection and deprotection of functional groups, which results in complex synthetic pathways and low yields of the final products. Enzymes, on the other hand, are brilliant at recognizing small differences in molecules. They can link two sugar compounds together in a highly specific manner. This property has been used to scale up synthesis of the disaccharide cellobiose and for the synthesis of complex human milk oligosaccharides (HMO) with up to five sugar building blocks (for more details, see Chapters 2.4 and 2.5 in Ref. 1).
HMOs are a complex and structurally diverse family of glycans that are found exclusively in humans. They are credited for many of the health benefits of breastfeeding. Infant formula producers have increased demand for HMO production at an industrial scale. To meet this need, Glycom and c-LEcta developed enzymes for the selective linkage of fucose or sialic acid to a tetrasaccharide in a single step, with no protective group chemistry or activation. It is impossible to catalyze this type of reaction with traditional chemical methods. Enzyme engineering was the key to enabling regioselective catalysis with a high yield of desired products (Figure 4).
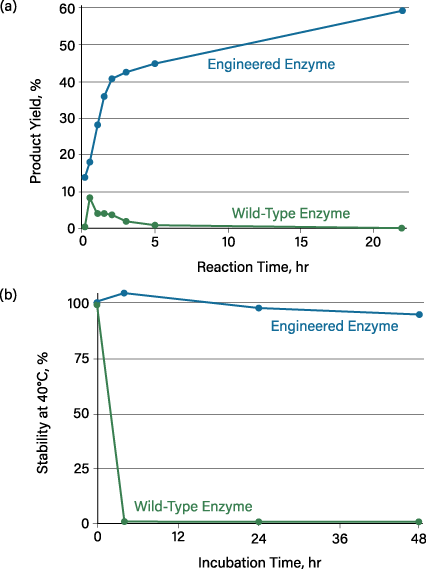
▲Figure 4. Engineered enzymes improve the enzymatic synthesis of complex human milk oligosaccharides (HMOs) for infant formula. (a) The reaction with the wild-type enzyme (green) produces a small amount of product in the first hours that is later destroyed by hydrolysis. The optimized variant (blue) eliminates the hydrolytic side reaction, leading to much higher product yield. (b) The wild-type enzyme rapidly loses activity at the desired process temperature, while the mutant is stable for 48 hr under the process conditions
Looking forward
Innovative engineers have used enzymes in industrial applications since their initial discovery. The implementation of evolutionary methods in enzyme engineering has expanded the range of industrial enzyme applications. Today, enzyme engineering is a mature field that can predictably optimize a catalyst for the synthesis of a desired product that performs effectively in an industrial process.
Bioinformatics has become more important in recent years to provide sophisticated design algorithms for variant libraries. While it is still necessary to develop enzymes in the lab, bioinformatics may become so powerful in the future that computers will be able to make predictions for the perfect enzyme, eliminating the need for screening large enzyme variant libraries. To arrive at this future scenario, we must work to understand the details of these complex high-performance athletes. Today’s methods of enzyme engineering can unleash the hidden potential of enzymes for even more applications — we just have to apply this potential.
Literature Cited
- Vogel, A., and O. M. Oliver, Eds., “Industrial Enzyme Applications,” John Wiley and Sons, Hoboken, NJ (2019).
- Rozzell, D., “Commercial Scale Biocatalysis: Myths and Realities,” Bioorganic and Medicinal Chemistry, 7 (10), pp. 2253–2261 (1999).
- Arnold, F. H., “Innovation by Evolution: Bringing New Chemistry to Life,” www.nobelprize.org/prizes/chemistry/2018/arnold/lecture (2018).
- Wu, S., et al., “Biocatalysis: Enzymatic Synthesis for Industrial Applications,” Angewandte Chemie, 60 (1), pp. 88–119 (2021).
Copyright Permissions
Would you like to reuse content from CEP Magazine? It’s easy to request permission to reuse content. Simply click here to connect instantly to licensing services, where you can choose from a list of options regarding how you would like to reuse the desired content and complete the transaction.