The scraped-surface freezer is at the core of ice cream production. Understanding and controlling this operation and the storage conditions of the final product are critical to creating the smooth and creamy treat consumers expect.
Ice cream is a multiphase soft solid composed of ice crystals, casein micelles, air cells, and emulsion droplets (both individual and clustered), all embodied within an unfrozen serum phase that contains dissolved components. These disparate parts interact as ice cream melts in the consumer’s mouth, which defines the overall quality or acceptability of the product. Controlling the formation of these different phases as the ice cream freezes requires an understanding of advanced engineering principles.
Although frozen desserts have existed for centuries, modern manufacturing processes employ advanced control to ensure efficient operation that creates products with desirable attributes. Building on the rudimentary freezers of yesteryears, the modern scraped-surface freezer (SSF) efficiently creates the structures necessary for frozen desserts. However, the inner workings of the freezer (i.e., a scraped-surface heat exchanger) remain somewhat elusive. This is in part because the inner workings are not visible for analytical measurement, but also because ice changes extremely rapidly as temperature varies.
In particular, controlling the creation of the ice phase is critical to product quality and shelf life. Numerous ice crystals smaller than about 50 μm produce a desirable smooth and creamy texture. The freezer must create these small ice crystals while also controlling the other phases (i.e., air and fat). The small ice crystals created in the freezer, however, are unstable; their high surface energy leads to recrystallization. To maintain the small ice crystals on the shelf, storage conditions must minimize recrystallization mechanisms, such as Ostwald ripening, in which small crystals dissolve and redeposit onto larger crystals.
Recent advances in the production of frozen desserts include developments of alternative processes and advances in modeling of freezers. This article provides a brief overview of ice cream production and the operation of the SSF and details the engineering behind these alternative processes and models of existing freezers.
Ice Cream or Frozen Dessert?
In the U.S., ice cream has a Standard of Identity as defined by the U.S. Food and Drug Administration (FDA) in the Code of Federal Regulations. It must contain no other fat than milk fat, and the minimum fat content is 10%. There is also a maximum density requirement that effectively caps the amount of air that can be incorporated at 100% overrun (air incorporation on a volume basis). If the requirements of the Standard of Identity are not met, the product cannot be called ice cream on the label, but instead must be called a frozen dessert.
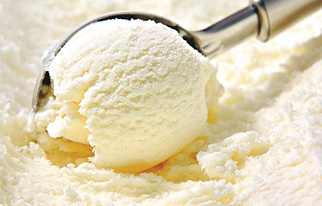
The traditional ice cream process
The process of making ice cream starts with creating a mix from the component ingredients. Cream, or some other dairy ingredient, and sugar are the main components that make up the bulk of the ice cream mix. A variety of ingredients that enhance the product or reduce cost may also be added. Emulsifiers are generally needed to control the emulsion interface. Stabilizers (e.g., hydrocolloids such as gelatin, gums, or cellulose) are often added to increase viscosity and extend shelf life. Other ingredients that may be added include corn syrup solids, skim milk solids, and, of course, colors and flavors. After freezing, variegates (e.g., caramel, marshmallow, fudge) and particulates (e.g., chocolate chips, cookie dough, nuts, fruits) may be added to provide unique characteristics.
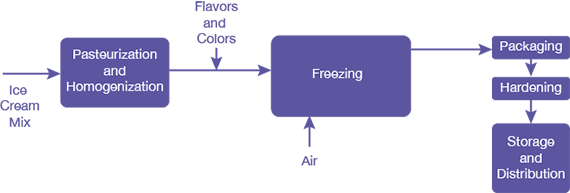
▲Figure 1. This simplified flow diagram depicts the steps of a continuous ice cream production process.
Making ice cream commercially involves a variety of unit operations (Figure 1). All of the ingredients are first combined to form the liquid mix. This mix is pasteurized to destroy pathogenic bacteria and then homogenized to ensure individual fat globules are about 1 μm. Colors and flavors are added to the liquid mix, after which it is frozen into ice cream in a continuous freezer. In the primary freezer, often called the dynamic freezing step, ice cream structures (e.g., ice crystals, air cells, clustered fat globules) are formed. The semi-fluid product of the dynamic freezing step is packaged before it enters a hardening tunnel or room, where secondary freezing takes place. After exiting the hardening tunnel, the product is ready to be shipped for distribution to consumers.
The quality of ice cream is highest immediately after manufacture, since storage conditions generally degrade product quality. Thus, controlling the dynamic freezing step is a key part of creating products with high quality and long shelf life.
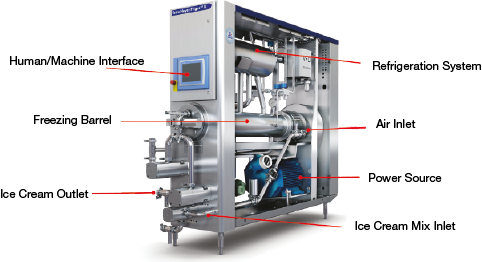
▲Figure 2. This cutaway diagram reveals the interior of a modern ice cream freezer. Image courtesy of Tetra Pak.
The dynamic freezing step occurs in a scraped-surface heat exchanger (Figure 2). The liquid mix enters the continuous freezer at about 4°C, where it is partially frozen, and exits as a semi-frozen slurry that resembles soft-serve ice cream. Air is incorporated into the mix and broken down into small air cells by shear forces within the freezer. During this process, individual fat globules (≈1 μm) begin to form clusters of partially coalesced fat globules of about 10–100 μm. These structures govern the ice cream’s quality and shelf life.
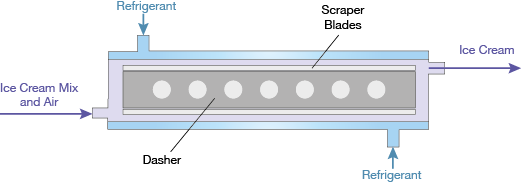
▲Figure 3. A rotating dasher with scraper blades clears product from the inside surface of the barrel, incorporating it into the bulk of the mixture.
Figure 3 details the freezing barrel of the SSF. Within the cylindrical barrel, a rotating dasher with scraper blades continually clears the inside surface of the barrel. Vaporizing refrigerant on the outside of the barrel cools the surface. Ice cream mix that enters one end of the barrel encounters this cold surface and begins to freeze at the wall. Ice continues to develop as the mix passes along the length of the barrel until it exits as a soft-serve slurry.
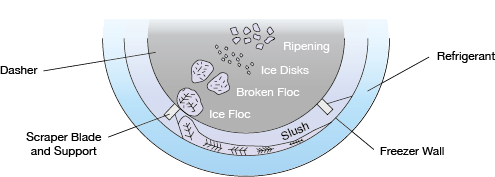
▲Figure 4. As ice crystals form, the scraper blade moves slush away from the freezer wall and incorporates it back into the bulk.
A dendritic ice slush forms on the inside of the barrel (Figure 4) because of the high driving force for freezing (i.e., large temperature difference between the mix freezing temperature and the wall temperature). The scraper blades clear the barrel surface approximately every 0.1 sec, depending on the dasher design and rotation speed. The cold ice slush mixes into the center of the barrel where temperatures are much warmer. The flow path is governed by the flow profile set by the interior dasher (or beater) design.
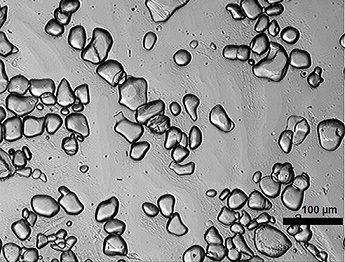
▲Figure 5. The ice crystals that exit an ice cream freezer typically measure 20–35 μm.
The average temperature decreases along the axial length of the freezer, approximately following the freezing point depression curve. Complex heat- and mass-transfer processes occur as ice melts and then recrystallizes, eventually creating a distribution of block-shaped ice crystals with a mean crystal size at the freezer exit of 20–35 μm (Figure 5). Crystal size varies based on operating conditions and mix formulation.
The ice cream slurry exits the freezer at about –6°C and is dispensed into containers. The choice of draw temperature weighs opposing goals: freezing as much as possible in the dynamic freezing step and ease of packaging. The more ice frozen during dynamic freezing, generally the smaller the ice crystals, since no other step in the process involves nucleation. However, a product with more ice will have a higher viscosity, which makes complete filling of containers problematic; an ice cream that is too stiff leaves air pockets as the product fills the container.
After packing, the containers of ice cream are sent to a hardening tunnel, where the temperature is about –30°C; there the temperature within the container is quickly reduced. Secondary freezing occurs as the ice crystals created in the dynamic freezing step increase in size based on the new phase equilibrium at the lower temperature. Crystals grow to about 35–45 μm after hardening, although this depends somewhat on the size and shape of the container. Product on the outside of the container freezes faster than that in the center, where there is a greater opportunity for ice crystals to grow larger through recrystallization mechanisms.
Cold extrusion processing
Cold extrusion is one of the most creative recent innovations in ice cream processing (1, 2). Instead of packaging ice cream after it exits the SSF, the slurry is pumped into a low-temperature extruder to reduce the temperature. The extruder quickly cools the slurry to about –12°C. The slow rotation of the extruder screw(s) provides uniform cooling and minimizes recrystallization of ice crystals and coalescence of air bubbles. The finished product has smaller ice crystals and air cells and, interestingly, still maintains sufficient fluidity to completely fill the package.
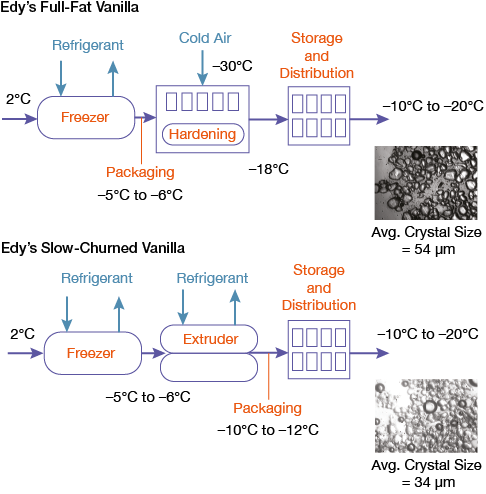
▲Figure 6. Ice cream produced through cold extrusion has significantly smaller ice crystals in the finished product (34 μm) than ice cream produced by traditional methods (54 μm).
Figure 6 compares a traditional ice cream process to the cold extrusion process. Marketers often call this “slow-churned” ice cream to differentiate it from the traditionally made products. Unfortunately, the cost of adding an extruder at the end of each SSF in commercial production limits the application of this new technology, despite its distinct advantages.
Modeling the freezer
Although computational fluid dynamics (CFD) modeling is widely used in other fields, its application to foods has been slow (3). CFD can provide a window into the complicated fluid dynamics within the enclosed SSF. The complex structure development changes the nature of the flow patterns, so CFD models must somehow account for the dynamic nature of the multiphase system.
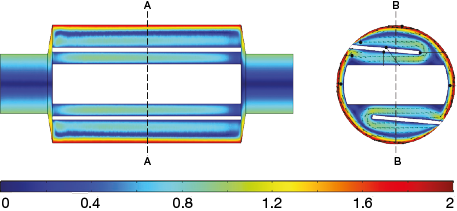
▲Figure 7. CFD modeling shows the velocity profile of sorbet inside a scraped-surface heat exchanger, assuming single-phase flow. Source: (4).
Hernandez-Parra et al. (4) recently modeled heat transfer and flow of sorbet in an SSF. Sorbet is a relatively simple model system because only ice forms, with no air incorporation or fat globule structuring. A key element in modeling sorbet is the change in fluid properties as the product transitions from a liquid mix to an ice slurry exiting the freezer. By assuming an ice phase equilibrium along the length of the freezer, they were able to develop correlations for viscosity (consistency coefficient for a shear-thinning fluid), specific heat capacity, and thermal conductivity along the length of the freezer. Assuming single-phase flow (i.e., neglecting ice effects on flow properties), they predicted a wide range of parameters, including shear rate profile, velocity profile (Figure 7), pressure profile, temperature profile, enthalpy and ice fraction along the freezer, and viscous dissipation rate. To validate the model, they compared measured temperature profiles to predicted values for a range of operating conditions, including dasher rotation speed, throughput rate, and refrigerant temperature. In all cases, predicted values agreed well with the experimental data, suggesting the model was accurately predicting conditions within the freezer.
The next step in modeling is to add modules that predict ice crystallization and air cell breakdown in the SSF. An early attempt to predict ice crystal size in an SSF by Arellano et al. (5) used a simple residence time distribution model coupled with a population balance approach. One of the problems with this approach is identifying the appropriate crystallization kinetics. Nucleation occurs on the barrel wall, followed by ripening and growth within the warmer center of the barrel. Kinetic expressions for these complex processes are generally not available. Thus, Arellano et al. used literature values where they could and resorted to setting some variables for optimization to fit the experimental size data. More work is needed to improve this approach. Similarly, Hernandez-Parra et al. (6) have attempted to couple CFD modeling with a capillary number approach to predict maximum air bubble size.
In the future, multiphase CFD modeling might be coupled with population balance models for both ice crystal and air cell size distributions to predict the product output. Such a model could enable simulations that inform freezer designs and predict operating conditions that produce desirable ice cream structures. The current market, which is flooded with new styles of frozen desserts (high air, high protein, low fat, low sugar, etc.) with little to no scientific underpinning, could benefit from this type of model.
Shelf life stability
Coarsening of ice crystals during storage is one of the main issues for frozen foods. Coarsening occurs due to the Gibbs-Thomson effect, in which there is a slight difference in melting point between small crystals and large crystals (7, 8). True Ostwald ripening, however, is quite rare in frozen desserts, mostly because other mechanisms of recrystallization occur more rapidly (8, 9). The close proximity of ice crystals in frozen desserts encourages rapid accretion, in which two adjacent crystals quickly form a neck and then gradually merge into one large spherical ice structure.
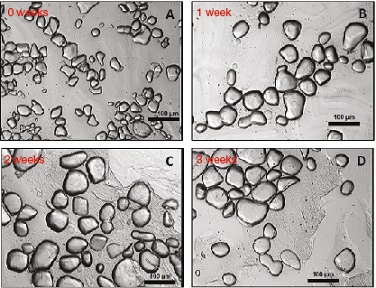
▲Figure 8. Ice crystals decrease in number and increase in mean size over a period of three weeks.
Furthermore, storage conditions for frozen desserts are not ideal. Temperatures fluctuate readily and rapidly, which causes melting and refreezing as the ice attempts to maintain a phase equilibrium. Over time, smaller crystals melt while larger ones grow. In general, this is the main mechanism for recrystallization since all freezers, especially frost-free freezers, undergo significant thermal cycling. Figure 8 illustrates the changes ice crystals undergo during three weeks of accelerated storage.
Although much work has been done to quantify the rates of recrystallization and the general mechanisms are well known, a comprehensive thermodynamic treatment of frozen desserts has not yet been done. Recently, van Westen and Groot (10) applied fundamental thermodynamic principles to model Ostwald ripening in frozen systems to predict ice crystal coarsening with time. Their results predicted experimental data reasonably well, although some adjustment of parameters was necessary. The model was then used to simulate the evolution of average ice crystal size with time. As models like these become increasingly accurate, they will be able to predict shelf life for a range of storage conditions, which could be tailored to extend shelf life. Such models could be expanded to account for the effects of various additive ingredients, particularly stabilizers, that are added to ice cream to inhibit recrystallization.
Closing thoughts
Despite centuries of ice cream production, the engineering principles have not yet been fully described, particularly those of the dynamic freezing step. The current drive for frozen dessert manufacturers to use fewer ingredients and provide more health-conscious options has revealed this lack of understanding. Some new products have suffered from commercial issues, such as collapse of high overrun products on the shelf. As a result, improving our understanding of the science that underlies the production of ice cream and frozen desserts should be a high priority.
Literature Cited
- Windhab, E. J., and S. Bolliger, “Low Temperature Ice Cream Extrusion Technology and Related Ice Cream Properties,” European Dairy Magazine,10, pp. 24–28 (1998).
- Wildmoser, H., “Impact of Low Temperature Extrusion Processing on Disperse Microstructure in Ice Cream Systems,” PhD Dissertation presented at the Swiss Federal Institute of Technology (ETH), Zurich, Switzerland (2004).
- Norton, T., and D. Sun, “CFD: An Innovative and Effective Design Tool for the Food Industry,” Aguilera et al., eds., Food Engineering Interfaces, Springer, New York, NY, pp. 45–68 (2011).
- Hernández-Parra, O. D., et al., “Modeling Flow and Heat Transfer in a Scraped Surface Heat Exchanger During the Production of Sorbet,” Journal of Food Engineering, 221, pp. 54–69 (2018).
- Arellano, M., et al., “Coupling Population Balance and Residence Time Distribution for the Ice Crystallization Modeling in a Scraped Surface Heat Exchanger,” Chemical Engineering Science,102, pp. 502–513 (2013).
- Hernández-Parra, O. D., et al., “Effect of Process Parameters on Ice Crystals and Air Bubbles Size Distributions of Sorbets in a Scraped Surface Heat Exchanger,” International Journal of Refrigeration, 92, pp. 225–234 (2018).
- Mullin, J. W., “Crystallization, 4th Ed.,” Butterworth-Heinemann, Oxford, U.K. (2001).
- Goff, H. D., and R. W. Hartel, “Ice Cream, 7th Ed.,” Springer, New York, NY (2013).
- Hartel, R. W., “Mechanisms and Kinetics of Recrystallization in Ice Cream,” The Properties of Water in Foods: ISOPOW 6, D. S. Reid, ed., Blackie, London, U.K., pp. 287–319 (1998).
- van Westen, T., and R. D. Groot, “Predicting the Kinetics of Ice Recrystallization in Aqueous Sugar Solutions,” Crystal Growth & Design,18, pp. 2405–2416 (2018).
Copyright Permissions
Would you like to reuse content from CEP Magazine? It’s easy to request permission to reuse content. Simply click here to connect instantly to licensing services, where you can choose from a list of options regarding how you would like to reuse the desired content and complete the transaction.