Although the wind and sun are free, converting these resources to useful power costs money. Renewable power needs to be deployed for CO2 mitigation where it can be used most efficiently and have the greatest benefit.
Global warming, often linked to increasing carbon dioxide in the atmosphere, is one of the major issues facing our planet (Figure 1) (1). As chemical engineers, we have a responsibility to implement technologies that meet our energy needs while minimizing emissions of carbon dioxide and other greenhouse gases. (Editor’s note: Read the CEP Special Issue “Thinking About Climate” for a thorough discussion of climate change.)
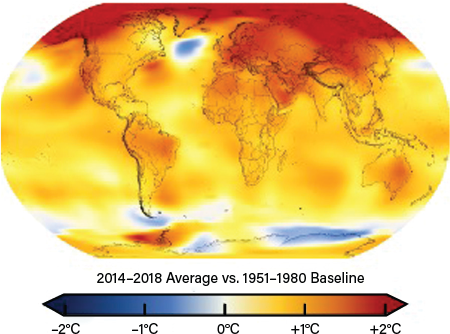
▲Figure 1. This map displays the differences in global surface temperatures between the five-year averages for 2014–2018 and the 30-year baseline period of 1951–1980. Higher-than-normal temperatures are shown in red and lower-than-normal are shown in blue (1).
Although various approaches are available, under development, or proposed to mitigate carbon dioxide emissions, few actual projects are in operation. Both capital and energy costs for mitigation technologies are high and, in the absence of a carbon tax or other government intervention, financial incentives are lacking. Engineers, scientists, and policymakers are in general agreement that the capital costs for these technologies must be reduced. In addition, as all of these technologies require energy, it is important to examine the energy requirements of the various mitigation solutions. Renewable energy is often viewed as a free resource, thus the energy efficiency of mitigation routes is not considered. However, energy in any form has value and it should be used as efficiently as possible to maximize its benefits. The use of renewable energy can help significantly lower carbon dioxide emissions, as well as prevent depletion of finite fossil resources.
This article discusses various methods to mitigate CO2 emissions that employ renewable power. It shows that avoiding CO2 emissions is the most efficient use of this energy, and the utilization of carbon dioxide for production of chemical or fuel products is the least efficient.
Carbon dioxide mitigation methods
Methods for carbon dioxide mitigation fall into one of three categories (Figure 2):
- Capture and sequester. Fossil carbon compounds from the Earth are burned to extract energy. The resulting carbon dioxide is captured and stored in some form (i.e., sequestered). Energy is required for capture, which can come from either additional fossil energy or from renewable sources.
- Capture and utilize. Fossil carbon compounds are burned to extract energy. The carbon dioxide is captured and converted into useful products. Energy is required for carbon capture, as well as for conversion to products.
- Avoidance. Renewable energy is used to displace energy obtained from burning carbon compounds, avoiding the production of carbon dioxide.
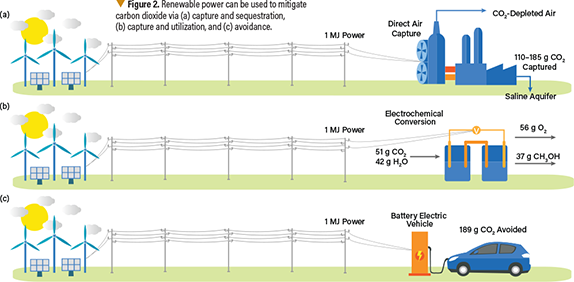
Evaluating the effectiveness of using renewable power for various mitigation methods requires a quantifiable estimate of their efficiency. In Ref. 2, thermodynamic arguments and established efficiencies are used to estimate the quantity of CO2 mitigated per MJ of renewable energy for various solutions (Table 1).
Table 1. Ranking of schemes that utilize renewable power to mitigate CO2 (2). | |
CO2 Mitigation Strategy | Mass CO2 Mitigated per Unit Renewable Energy, g CO2/MJ |
Renewable power displaces coal-fired power plants | 291 |
Renewable power is distributed through the grid to electric vehicles (EVs), displacing internal combustion engine vehicles (ICEVs) | 189 |
Renewable power is used for direct air capture (DAC) of CO2 | 110–185 |
Renewable power displaces natural gas-fired open cycle power plants | 141 |
Renewable power is used for the electrolysis of water, producing H2 for use in fuel cell vehicles (FCVs) | 103 |
Renewable power is used for the electrolysis of water, producing H2, which is used to thermocatalytically convert CO2 to methanol, displacing gasoline in ICEVs | 51 |
Capture and sequestration
Humans have been converting fossil energy sources into useful work and heat for hundreds of years; these fossil energy sources emit carbon dioxide when combusted, which accumulates in the atmosphere. Various strategies can be used to capture CO2, allowing the carbon to be returned to the Earth. Methods of CO2 capture can be classified as either post- or pre-combustion: Post-combustion capture refers to removal of CO2 after complete combustion of the hydrocarbon feedstock, typically at low (atmospheric) pressure; pre-combustion capture refers to processes where CO2 is removed before complete combustion of the hydrocarbons.
Two large-scale projects have been built to capture carbon dioxide in the fluegas of coal-fired power plants: Boundary Dam in Saskatchewan, Canada, and Petra Nova in Texas (Figure 3) (3). These projects were justified based on projected profits from selling the CO2 for enhanced oil recovery (EOR). However, these projects require oil prices to be high to ensure profitability, and they are unprofitable at the current low prices. The energy for the capture operation is taken from the power plant itself, which decreases the plant’s total power output by about 27% (4). For coal-fired projects, carbon capture and sequestration is highly efficient, capturing 800–900 g CO2 per MJ energy input. However, the capital costs are large, essentially derating the power plant by 27%.
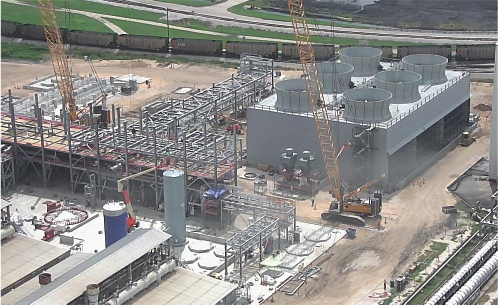
▲Figure 3. The Petra Nova carbon capture and sequestration project is designed to capture approximately 90% of the CO2 from a 240-MW slipstream of fluegas and use or sequester approximately 1.4 million m.t. of greenhouse gas per year. Image and data courtesy of the U.S. Dept. of Energy (DOE).
The chemical process industries (CPI) already practice pre-combustion capture of carbon dioxide. In processes to produce hydrogen, ammonia, ethylene oxide, and methanol, CO2 is produced as a byproduct at pressures high enough to enable its recovery. However, most of this captured CO2 is not yet sequestered or otherwise utilized, representing a low-cost opportunity to mitigate modest quantities of carbon.
Pre-combustion capture can also be done using an integrated gasification combined cycle (IGCC), which uses a high-pressure gasifier to turn coal and other carbon-based fuels into pressurized syngas. Carbon can be removed from the syngas prior to combustion of the hydrogen in a gas turbine.
Capture and sequestration could curb increases in global atmospheric CO2 concentration, but direct air capture (DAC) is the only method that can directly reduce the current concentration. While DAC is not carried out on any significant scale today, many feasible schemes have been proposed and investment in the technology is growing (5). DAC systems all involve absorption or adsorption of atmospheric CO2 via a series of chemical reactions. Reagents are then regenerated, releasing the relatively pure carbon dioxide and enabling capture and containment.
Energy is required to regenerate the reagent, as well as to move vast quantities of air through the process. Due to the very dilute concentration of CO2 in air (~400 ppm), DAC would require at least three times as much energy as capture from fluegas (which is about 10–14% CO2), and even more would be required to move air through the process.
Carbon Engineering, a Canada-based clean energy company, has developed a process for DAC. Their website claims that their process captures about 185 g CO2 per MJ energy input (6). The International Energy Agency (IEA) estimates lower capture efficiencies of 110–150 g CO2 per MJ (7).
Renewable power, such as wind and solar, could be directly coupled to DAC plants in remote locations. The DAC plant would inevitably operate at less than 100% capacity, as carbon dioxide would only be captured when the renewable energy source is available (e.g., when the wind blows or the sun shines).
Carbon dioxide utilization
An alternative to sequestering captured carbon is converting it to useful products, such as fuels, chemicals, or inorganic materials. Carbon dioxide utilization (referred to as CCU, CDU, or carbon recycling) could enable the removal of CO2 from the environment while generating revenue, a seemingly ideal solution for the CPI.
In practice, however, the barriers to CO2 utilization are quite high (8). The basic and unavoidable hurdle is that it requires the input of large amounts of energy to turn carbon dioxide into something useful. Most CO2 is produced when energy is extracted by combustion. The first law of thermodynamics requires that the same amount of energy must be added as extracted to return it to a high-energy state. A net reduction in emissions is unlikely if fossil energy is used for CO2 utilization. For this reason, most schemes envision the addition of renewable energy, with many proposing the use of hydrogen obtained from the electrolysis of water using renewable electricity and others utilizing the renewable electricity directly via electrochemical reduction of CO2.
If CO2 utilization is carried out using renewable energy, CO2 only acts as an energy carrier in a considerably energy-inefficient and capital cost-intensive process. Other CO2 mitigation approaches will almost always be less expensive and a better use of limited renewable energy. For example, if renewable energy were used to produce hydrogen for the thermocatalytic conversion of CO2 to methanol, Table 1 states that 51 g of CO2 could be converted to methanol for every MJ of renewable energy input. If the same amount of hydrogen was used to power an electric vehicle, it would save 189 g of CO2 per MJ energy from being emitted. This comparison does not even consider the large amount of capital required for electrolysis and methanol synthesis.
For the foreseeable future, CO2 utilization will likely only be practical in limited circumstances. It may make sense, for example, to convert carbon dioxide to an easily transportable product, enabling export of otherwise stranded energy. Carbon Recycling International’s (CRI’s) Icelandic methanol plant takes advantage of local geothermal energy to upgrade CO2 to methanol, which can be easily transported (9). Carbon recycling may also be practical if process efficiencies can be gained by using CO2 or if CO2 is necessary for the synthesis of a product (e.g., novel CO2-containing polyols) (10). In such cases, however, careful analysis is required to ensure that the amount of CO2 conversion justifies the amount of energy consumed, and the resulting gains, if any, are likely to be small. As in DAC, a renewable source such as wind and/or solar could be directly coupled to the CO2 utilization plant, but this will result in low utilization of the expensive capital asset.
Carbon dioxide avoidance
An obvious approach to carbon dioxide mitigation is simply to limit the amount made in the first place. Processes can be made more energy efficient or carbon-rich fuels and feedstocks (e.g., coal, crude oil, naphtha) can be replaced with hydrogen-rich sources (e.g., methane, ethane, propane). The greatest opportunity, however, is to use renewable energy for power production and transportation.
Renewable energy has already significantly penetrated the electric grid. Wind and solar accounted for about 9% of U.S. power production in 2019. Hydropower accounts for an additional 7% of the electric grid, but this technology is mature and not likely to further displace fossil power production (11).
The power plant fuel source has a significant impact on the amount of CO2 emissions that can be avoided by switching to renewable sources; displacing coal with renewables has the biggest impact on carbon emissions, mitigating 291 g CO2 per MJ renewable energy. Replacing a natural gas-fired plant with renewables mitigates about 99–141 g CO2 per MJ renewable energy.
Due to the variable nature of renewable power, the extent to which it can displace fossil power is limited. Increasing the renewable share of grid power requires grid storage and flexible loads (12). Grid energy storage, such as pumped hydro, batteries, and thermal and mechanical methods, can be quite costly (13). Flexible loads are suitable for use with renewables because they allow power to be consumed when supply is in excess. The CPI have relatively constant power demand, but new technologies may enable a variable structure. For example, energy could be stored as cold water or ice when power supply is in excess and used for process chilling during peak power demand (14).
Electric vehicles (EVs), either battery electric or plug-in hybrid, offer another route to flexible power loads. Vehicle-to-grid (V2G) power technology can be used to allow a smart grid to balance supply and demand by controlling the flow of power to or from the EV (15). In its simplest form, one-way V2G charges the vehicle at night when power demand is low and supply (e.g., wind) is high.
In addition to helping match demand with supply from variable sources, EVs also avoid CO2 emissions when they displace internal combustion engine vehicles (ICEVs). This appears to be the most effective use of renewable power for mitigating CO2. If an EV displaces an ICEV, it mitigates 189 g CO2 per MJ renewable energy (2). DAC, on the other hand, mitigates 110–185 g CO2 per MJ renewable energy. In addition, EVs have two significant advantages over DAC:
- the capital cost of DAC plants will be significant, while the cost of an EV is basically the difference in cost between an EV and an ICEV
- DAC does not help balance variable supply with demand — the capacity of the DAC plant cannot be fully utilized on renewable supply alone.
As an alternative to EVs, renewable power can be used to electrolyze water to hydrogen, which can be used to power hydrogen fuel cell vehicles (FCVs). This is a less effective use of renewable power for CO2 mitigation than an EV, mitigating 103 g CO2 per MJ renewable energy (2). While this does allow storage of energy in the form of hydrogen, electrolysis plants have high capital costs and can only operate when renewable supply is available.
In the CPI, opportunities are beginning to appear for electrification, replacing fossil fuel-derived energy with electric power. The technology is already available to electrify pumps, compressors, steam generation, and many low-temperature heating operations. Development is underway for more challenging applications, such as electrifying high-temperature furnaces.
While this does not address the issue of variable renewable power, electrification can reduce CO2 emissions if the power supply is sufficiently efficient and/or if a suitable portion of the power is supplied by renewables. For example, using a boiler and condensing turbine to supply shaft power is about 35% efficient. If the efficiency of fossil fuel electricity is higher than 35%, then replacing the steam turbines with electric motors can produce a net reduction in CO2 emissions (assuming the same fuel source in both cases). Similarly, for heating applications at modest temperatures, a heat pump can convert a given amount of power into three times as much heat. Replacing fossil fuel heaters at modest temperatures with heat pumps can decrease CO2 emissions if electricity is available from a high-efficiency or renewable source.
Carbon dioxide mitigation per unit renewable energy can vary widely from process to process, but initial estimates suggest potential savings of 50–130 g CO2 per MJ for full process electrification and up to 200 g per MJ for some partial electrification options. Deployment on a large scale has been limited due to the generally higher energy costs for electric unit operations, but electrification will likely become more widespread if financial penalties for carbon emissions increase.
Lessons for the CPI
Solving global problems associated with carbon dioxide accumulation in the atmosphere requires a two-pronged approach: reducing our energy requirements and converting our energy supply from fossil to renewable sources. While the wind and sun are free resources that are virtually unlimited, there are significant costs to converting this energy to useful power. Renewable power for CO2 mitigation should be deployed where it can be used most efficiently and will have the greatest impact on CO2 emissions.
While many approaches are available to use renewable power to mitigate CO2 emissions, renewable power is most efficiently used when it helps to prevent CO2 emissions (e.g., replacing ICEVs with EVs). The CPI can contribute to this space by advancing technologies that enable the efficient use of renewable power, such as improving battery manufacturing and recycling and enabling smart grid and V2G technology to help address the supply-demand imbalance inherent with renewable energy.
As financial penalties for CO2 emissions rise and the amount of renewable energy on the grid increases, the CPI should search for cost-effective ways to replace energy derived from fossil fuels with electrical energy. Efficient applications for electricity such as electric motors and heat pumps are the most promising short-term opportunities. As the world transitions to greater dependence on renewables, the CPI should consider more significant process modifications, including more efficient use of electrical power and technologies that enable flexible power demand.
DAC coupled with renewable power can enable further reductions in CO2, but the captured CO2 must be sequestered efficiently and for a sufficiently long time. The experience and resources of the CPI can help to optimize processes for DAC and advance sequestration technologies.
Utilization of CO2 to produce useful products is the least efficient use of renewable power for CO2 mitigation. It is not recommended to deploy resources in the CPI to enable or develop CO2 utilization technologies to produce fuels or commodity petrochemicals.
While this article focuses on using renewable energy to mitigate CO2, the CPI can take steps to reduce CO2 emissions that do not require renewable energy, including improving the energy efficiency of existing processes. Evaluating process energy efficiency is probably the fastest and surest method of realizing some CO2 savings, especially if financial penalties on CO2 emissions incentivize equipment upgrades and process modifications. Switching to fuels that produce less CO2 per unit energy produced (e.g., replacing coal or heavy fuel oil with natural gas) is another near-term route to decreasing emissions. Capturing and sequestering CO2 produced within chemical processes is also an important, though modest, step the CPI can take. In addition, it is critical to improve the efficiency of processes to produce materials involved in the production, transmission, and use of renewable energy (e.g., solar panels, battery components, and lightweight automotive materials).
Literature Cited
- NASA Scientific Visualization Studio, “Global Temperature Anomalies from 1880–2019,” NASA, Washington, DC, https://svs.gsfc.nasa.gov/4787 (accessed Sept. 29, 2020).
- Lattner, J. R., “Carbon Dioxide Mitigation Using Renewable Power,” Current Opinion in Chemical Engineering, 29, pp. 51–58 (Sept. 2020).
- International Energy Agency, “CCUS in Power,” IEA, Paris, France, www.iea.org/reports/ccus-in-power (accessed Sept. 2020).
- He, X., and M.-B. Hagg, “Energy Efficient Process for CO2 Capture from Flue Gas with Novel Fixed-Site-Carrier Membranes,” Energy Procedia, 63, pp. 174–175 (2014).
- Sanz-Perez, E. S., et al., “Direct Capture of CO2 from Ambient Air,” Chemical Reviews, 116, pp. 11840–11876 (2016).
- Carbon Engineering, “Carbon Engineering,” https://carbonengineering.com/our-technology (accessed Sept. 29, 2020).
- International Energy Agency, “Direct Air Capture,” IEA, Paris, France, https://www.iea.org/reports/direct-air-capture (accessed Sept. 25, 2020).
- Stevenson, S. A., “Thermodynamic Considerations in CO2 Utilization,” AIChE Journal, 65 (9) (Sept. 2019).
- Chemicals Technology, “George Olah CO2 to Renewable Methanol Plant,” www.chemicals-technology.com/projects/george-olah-renewable-methanol-plant-iceland (accessed Sept. 29, 2020).
- Plastics Today, “Covestro Opens Industrial Scale CO2-based Polyol Plant,” Plastics Today, https://www.plasticstoday.com/materials/covestro-opens-industrial-scale-co2-based-polyol-plant (accessed Sept. 27, 2020).
- U.S. Energy Information Administration, “What is U.S. Electricity Generation by Energy Source?,” EIA, Washington, DC, https://www.eia.gov/tools/faqs/faq.php?id=427&t=3 (2019).
- Mai, T., et al., “Renewable Electricity Futures Study,” NREL, https://www.nrel.gov/analysis/re-futures.html (2012).
- Gur, T. M., “Review of Electrical Energy Storage Technologies,” Energy and Environmental Science, 11, pp. 2696–2767 (2018).
- Todd, F., “How Electro Thermo Energy Storage Could Redefine Sector Coupling,” NS Energy, https://www.nsenergybusiness.com/features/electro-thermal-energy-storage-sector-coupling (July 10, 2019).
- Lund, H., and W. Kempton, “Integration of Renewable Energy into the Transport and Electricity Sectors Through V2G,” Energy Policy, 26 (9), pp. 3578–3587 (2008).
Copyright Permissions
Would you like to reuse content from CEP Magazine? It’s easy to request permission to reuse content. Simply click here to connect instantly to licensing services, where you can choose from a list of options regarding how you would like to reuse the desired content and complete the transaction.