Sections
Commercial carbon capture and sequestration projects will handle very large quantities of carbon dioxide, which will present new challenges and hazards.
Carbon dioxide (CO2) capture, transportation, injection, and geological storage (CCS) requires the linking and integration of separate process activities to capture CO2 at an emitter facility, transport it to a designated storage site, and then inject it deep underground for permanent geological storage. The amount of CO2 handled within a commercial CCS operation may be in the order of thousands of tons per hour, with transportation systems likely to have inventories that are ten times more. CCS operations may range in complexity from relatively simple point-to-point chains with a single capture facility, storage site, and interconnecting pipeline, to multiple capture plants feeding CO2 into a network of pipelines from which the CO2 is supplied to multiple storage sites.
Many aspects of CCS have been successfully deployed in various industries; therefore, a wealth of experience is available. The challenge facing the emerging CCS industry is to take existing and new technologies, experience, and practices, ensure their suitability for use in CCS, and then integrate the various links in the CCS chain together to create an efficient, optimized, and safe operation.
CO2 has unique properties that must be understood and managed when handling such large quantities in different systems and locations. This article explores the potential hazards posed by large-scale CO2 releases (including the inhalation effects), and addresses the phase behavior of CO2 and the potential impact of impurities. Guidance is presented to raise awareness of important CO2 properties that may lead to or contribute to major accident events.
CO2 characteristics
CO2 is a colorless and odorless gas that is about 1.5 times heavier than air (at standard temperature and pressure conditions). Depending on the pressure and temperature of the system, CO2 can exist as a gas, liquid, solid, or supercritical fluid. Under normal atmospheric pressures, CO2 can only exist as a gas or solid; it cannot exist as a liquid under atmospheric conditions.
CO2 is fully oxidized and thus not very reactive nor flammable. Nevertheless, it poses a toxic and asphyxiation hazard. In humans, CO2 is a normal component of blood gases at low concentrations; however, if inhaled at certain concentrations, it can be lethal. Humans are sensitive to changes in CO2 concentrations.
In addition to the asphyxiation hazard of CO2 displacing oxygen in the air, the inhalation of elevated concentrations of CO2 can increase the acidity of the blood, triggering adverse effects on the respiratory, cardiovascular, and central nervous systems. A CO2 concentration of around 5% by volume in air may cause headaches, dizziness, increased blood pressure, and difficulty breathing within a few minutes. If concentrations above 17% by volume in air are inhaled, this could cause loss of purposeful activity, unconsciousness, convulsions, coma, and death within one minute. CO2 does not meet the definition of acutely toxic according to the Global Harmonized System (GHS) (1). However, it may be considered mildly toxic given the aforementioned hazards.
Professionals such as divers, submariners, astronauts, and anesthetists know very well the dangers of breathing high concentrations of CO2 since they need to maintain inhaled gas mixtures within acceptable limits to sustain life.
Human exposure limits are defined by regulators and are typically a function of concentration and exposure time. The U.S. Occupational Safety and Health Administration (OSHA) set an 8-hr exposure limit of CO2 to 5,000 ppm (0.5% by volume), i.e., permissible exposure limit (PEL) (2). The National Institute for Occupational Safety and Health (NIOSH) established an immediately dangerous to life or health (IDLH) CO2 concentration of 40,000 ppm (4% by volume) (3). It should be noted that the typical CO2 concentration outdoors is 300–400 ppm (0.03% to 0.04%), but this can be as high as 600–900 ppm in metropolitan areas (4).
With the development of CCS systems, where pipelines transport large inventories of liquid-phase or supercritical CO2 (in the order of tens to hundreds of thousands of tons), effective risk management of industrial systems handling large quantities of CO2 and a public understanding of the impact of CO2 on the human body is needed.
Past incidents
In the past, CO2 releases from different types of installations, including pipelines, have caused injuries and fatalities. The following discussions review some CO2 incidents that highlight the hazards when a large quantity of CO2 is released.
Satartia, MS (2020). A 24-in. buried pipeline transporting liquefied CO2 ruptured near Satartia, MS. A girth weld failure generated a CO2 plume that traveled toward surrounding populations. Emergency evacuation protocols were activated and approximately 200 residents were evacuated. There were no fatalities, but 45 people sought medical attention. The pipeline failed on a steep embankment. Heavy rains caused soil subsidence, creating axial strain on the pipeline that resulted in a full-bore rupture girth weld failure. The U.S. Pipeline and Hazardous Materials Safety Administration (PHMSA) investigation reported several contributing factors to the incident, including not addressing the risks of geohazards in plans and procedures, underestimating the potentially affected areas in the dispersion model, and not notifying local responders about a potential failure of the system (5).
Mönchengladbach, Germany (2008). Approximately 15 tons of CO2 were accidentally released from a fire extinguishing facility in Mönchengladbach, Germany. The released CO2 was not contained by the building due to the failure of a door to close, and CO2 spread outside where the air conditions were very stable with little wind. Although no fatalities were associated with this incident, 107 people were affected (19 of whom were hospitalized). Because CO2 is invisible, it was difficult for first responders to assess the extent of the hazard.
U.S. Environmental Protection Agency (EPA) database on fire protection systems. CO2 is one of the most used inert gas extinguishing agents. The EPA performed a comprehensive review of CO2 incidents related to its use in fire protection (6). From 1975 to 2000, a total of 51 incidents reported 72 deaths and 145 injuries from CO2 releases from fire extinguishing systems. This study reported that most of the incidents occurred during maintenance on or near the CO2 fire protection system. It is worth highlighting that the mass of CO2 to be released by a fire suppression system will depend on the area being covered, but would likely be in the order of tons.
Worms, Germany (1988). In 1988, a 30-m.t. capacity CO2 tank in Worms, Germany had a catastrophic vessel failure resulting from a cold CO2 boiling liquid expanding vapor explosion (BLEVE). The tank shattered, and tank fragments were propelled 300 m, resulting in three fatalities and extensive damage (7).
These release incidents show the potential consequences when CO2 forms a hazardous cloud and is inhaled by people and animals. It should be noted that the consequences typically associated with any type of release are very dependent on the source of the release (including the operating conditions of the system), the inventory released, the arrangement of the surroundings (e.g., surrounding populations), the ground topography, the weather conditions, potential containment by buildings or enclosed spaces, and other factors.
CO2 storage and transportation conditions
Figure 1 shows that pure CO2 can exist as vapor, liquid, solid, or supercritical fluid depending on the pressure and temperature (8, 9). At the triple point, the solid, vapor, and liquid phases coexist at equilibrium. Above the critical point, the system will be in supercritical condition. Supercritical CO2, also referred to as dense-phase CO2, has a viscosity similar to gas but a density closer to a liquid.
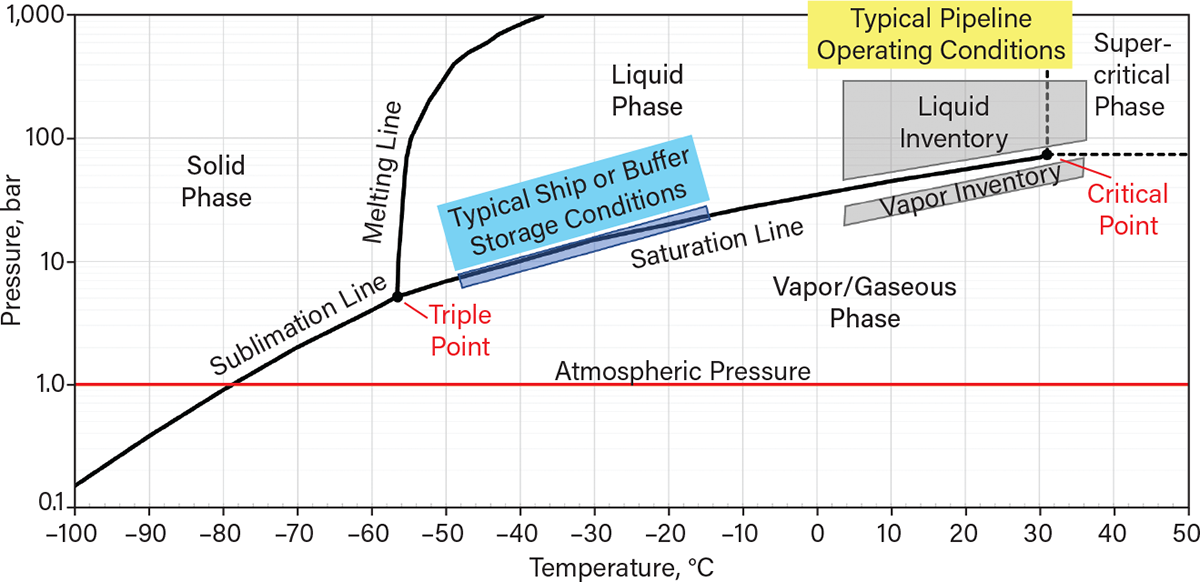
▲Figure 1. This CO2 phase diagram highlights typical transportation conditions. The red horizontal line represents atmospheric conditions at 1 bar (14.5 psi). Source: Adapted from (8, 9).
The horizontal line in Figure 1 represents atmospheric conditions at 1 bar (14.5 psi). It is observed that at atmospheric pressure, pure CO2 can only exist as a vapor or as a solid. And depending on the temperature, it will change phases directly between solid and vapor. At atmospheric pressure, the phase diagram shows that CO2 cannot exist as a liquid.
For CCS applications, CO2 is typically transported in pipelines or ships. To avoid two-phase flow within pipelines, CO2 is transported as a vapor, liquid, or supercritical fluid. In the case of ship transportation, CO2 is transported in storage tanks at a pressure and temperature on the saturation line; at this condition, the CO2 inventory is characterized by a liquid with a vapor space on top, both phases in thermodynamic equilibrium. Typical CO2 transportation conditions are illustrated in Figure 1. One of the main takeaways from the pure CO2 phase diagram is that the triple point and/or the critical point may be within or near the operating region of typical industrial processes and CO2 transportation systems.
Depressurization behavior. The phase diagram in Figure 2 shows what occurs when a pipeline or a storage system containing liquefied CO2 ruptures (8, 9). Assuming an inventory of liquid CO2 at 100 bar and 10°C (i.e., Point A), a breach to atmospheric conditions would cause the system to undergo a series of phase transitions represented by the red path from Point A (liquid phase at typical operating conditions) to Point B (vapor phase at ambient pressure).
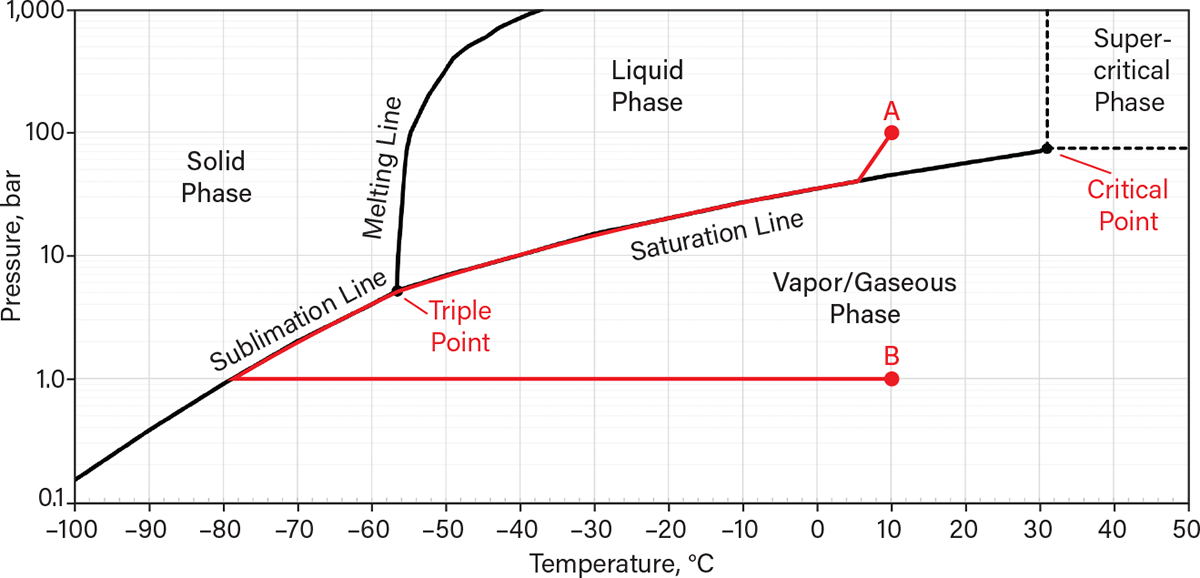
▲Figure 2. In this example, Point A represents an inventory of pure CO2 at 100 bar and 10°C (i.e., liquid-phase CO2 at typical operating conditions). If a rupture occurs, the CO2 will undergo a series of phase transitions to depressurize along the red path, eventually reaching Point B (vapor-phase CO2 at ambient conditions). Source: Adapted from (8, 9).
First, the pressure and temperature will decrease until reaching the saturation line. At this point, the phase transition starts and two phases (liquid and vapor) will coexist at equilibrium. The depressurization continues through the saturation line with the vaporization of the liquid.
Below the triple point, the liquid has transitioned into a mixture of vapor and solids, represented by the sublimation line. After reaching atmospheric pressure at 1 bar (14.5 psi), these solids will slowly sublime using the heat of the surroundings until reaching the final condition, corresponding to 100% vapor at ambient pressure and temperature. One of the most important takeaways from Figure 2 is the formation of solid CO2 (i.e., dry ice) during the depressurization process.
In general, two types of CO2 releases are associated with typical transport or storage systems: cold CO2 releases and hot supercritical releases.
A cold release corresponds to the depressurization example in Figure 2, where the initial operating conditions correspond to CO2 transported or stored as a liquid. Once system rupture occurs, the release behavior is characterized by a white cloud containing a mixture of vapor and solid CO2. It is important to recall that vapor CO2 is not visible; therefore, the CO2 cloud becomes visible due to the condensed water vapor formed by the humidity of the air combined with the cold temperatures upon release (below the dew point of the air). In this type of release, it is very common to observe deposits of solid CO2 on the ground surrounding the release point; this dry ice slowly transforms into vapor CO2.
The second main release type, commonly known as hot supercritical release, is typically associated with operating conditions in the supercritical region of the phase diagram, e.g., 150 bar (2,176 psi) and 150°C (302°F). In these releases, the formation of a visible cloud containing condensed water vapor does not occur since the temperature close to the release point is above the dew point of the air. The formation of solid CO2 does not take place during the release event since there is a direct transition from the supercritical to the vapor phase.
It is important to highlight that the CO2 release behavior varies significantly depending on the initial operating conditions in the system that ruptures. Therefore, it is recommended to use a temperature-entropy diagram to predict the potential release behavior and phase transition (if any) for the specific initial conditions of any industrial system storing or transporting CO2.
CCS facilities
A CCS chain may consist of some, or possibly all, of the following elements:
- CO2 capture facilities
- onshore pipeline
- underwater pipeline
- CO2 carrier ship and (un)loading facilities
- intermediate storage facilities
- injection facilities
- injection and other wells
- storage sites.
Comprehensive and detailed descriptions of the CCS chain are available in the Intergovernmental Panel on Climate Change (IPCC) special report on Carbon Dioxide Capture and Storage (10). The following subsections briefly discuss a few of the special issues and hazards to consider for various CCS facilities.
Capture facilities. The composition of the CO2 stream is dependent on the source and capture process. The source stream is unlikely to be 100% CO2 and will contain other components and impurities, such as carbon monoxide (CO), oxygen, water, argon, nitrogen, hydrogen, methane (CH4), hydrogen sulfide (H2S), sulfur dioxide (SO2), and heavy metals. These additional components have their own hazards and design considerations that should be accounted for. If multiple capture facilities are feeding into a common network, then a variety of compositions could potentially exist.
Impurities have several impacts on CO2 systems. The presence of impurities within the CO2 stream can change the phase envelope by introducing a two-phase liquid/vapor region and/or significantly raising or lowering the saturation pressure of the mixture. Impurities increase the mixture’s critical pressure and generally reduce the critical temperature; however, SO2 and H2S impurities cause an increase in critical temperature (11). Impurities vary significantly between CO2 sources, changing the properties and behavior of the CO2 stream and potentially impacting the system’s mechanical integrity.
Free water in the CO2 system can cause internal corrosion. The water can combine with CO2 to form carbonic acid, which is corrosive to carbon steels. Water content in the CO2 stream should be controlled to minimize the potential corrosion rate, and a robust inspection program should be implemented.
Pipeline transport. Following capture, the CO2 will need to be transported to handling or injection facilities. The CO2 will be transported via pipeline in vapor phase or as a high-density fluid. Corrosion concerns from the stream impurities are relevant for the pipeline integrity given the potential for cross-chemical reactions.
Supercritical CO2 is an excellent solvent that can break down lubricants. Any impurities within the stream solution will precipitate out at the point of pressure drop with a decline in solubility. For example, any heavy metals will precipitate out at a point of loss of containment and may pose a health or environmental hazard. CO2 is also highly invasive and can dissolve into some elastomers (e.g., seals), causing damage. Careful consideration when selecting lubricants and materials is necessary to ensure suitability for service.
Propagating pipeline fractures can release a large volume from the pipeline within a short period. The two fracture failure mechanisms — brittle and ductile — should be accounted for in the design. A brittle fracture occurs without (or with very little) plastic deformation of the material prior to failure; examples of pipes that may experience brittle fracture are those that operate at low temperatures or have poor microstructures. A ductile fracture is associated with large plastic deformation of the material prior to failure. Most pipe failures occur in a ductile manner.
Pipeline designs avoid propagating brittle cracks, but the potential low-temperature effects of a CO2 release should be accounted for in the design. Running ductile fractures must also be evaluated as part of the design, with fracture-arrest requirements (e.g., pipeline toughness, thickness) added as needed (12).
Injection. Injection facilities may need pressure-boosting or reduction systems depending on the nature of the transported CO2 and the characteristics of the storage/injection site. The design of wells for CO2 injection will be similar to those used for gas injection into current hydrocarbon fields. The design will still need to account for pressure, temperature conditions, and material selection to limit corrosion.
In the event of a well blowout, the release may contain downhole formation fluids and other components. The potential components in a formation release should be considered during design and hazard analysis.
General considerations. Whether by design or by accident, depressurization of CO2 can result in temperatures at or below –78°C (–108°F) within systems and/or within any release plume. In addition, significant quantities of solid CO2 can be formed within systems and/or within the release area, which could add to the low-temperature issue and cause system blockages. Releases within enclosed areas may experience a rapid temperature drop and significant solid particle formation. The impacts of low-temperature exposure should be accounted for in the system design and operation.
CO2 density is sensitive to temperature changes, especially close to critical point conditions (i.e., 31°C and 74 bar). This can lead directly to system over-pressurization with a relatively small change in CO2 temperature. Appropriate design of pressure relief systems should avoid this over-pressurization event.
Hazard management
Commercial CCS projects will handle very large quantities of CO2. Handling such large inventories of CO2 is new to many involved with CCS implementation. It is worth highlighting that regulatory frameworks are in place for CO2 pipeline systems. The hazard potential of CO2 must be managed along with the other potential hazards. Existing hazard management processes are suitable to manage these hazards if appropriately implemented. Generic approaches are described in International Organization for Standardization (ISO) 31000 (13) and “Guidelines for Risk Based Process Safety” (14). The risk management framework should implement the “Plan-Do-Check-Act” cycle.
Over the past decade, DNV has collaborated on joint industry projects and large-scale testing to understand the potential hazards of CO2. The company developed the CO2RISKMAN project and guidance as a robust knowledge source for CCS projects to understand CO2 hazards within the facility hazard management (8). This guidance is not prescriptive but is meant to provide the base information for operators to develop suitable hazard management strategies. When designing and operating CCS systems, keep the following guidelines in mind:
- Recognize the CO2 hazard potential through the application of robust hazard identification techniques and risk management systems.
- CO2 is a colorless and odorless gas at atmospheric conditions. A CCS CO2 release will be highly concentrated until dispersed. Before diluted to a concentration below 5% by volume in air, the plume will pose a hazard to people. CO2 is heavier than air and will accumulate in low areas. Managing the risks associated with hazardous mixture inhalation is well understood in industrial and public settings. This experience can be used to help ensure the safety of CO2 systems.
- Recognize that CO2 concentration associated with a release cannot be assessed by only looking at the size of the visible cloud. CO2 vapor is invisible — the visible cloud that is commonly seen is the condensing water vapor generated by the low temperatures upon release and is not representative of the CO2 concentration.
- Stream composition with all potential impurities needs to be documented and considered in the design. The CO2 stream specification should be defined with requirements for purification and drying to limit the potential impurities that promote internal corrosion. System monitoring should be in place to ensure that excursions outside the specification cannot occur.
- Use models for the prediction of release discharge conditions based on the potential stream composition and phase envelopes.
- Selection of materials is extremely important for seals, flexible hoses, instruments, wire and cable insulators, controls, and other safety-critical components.
- Pipeline designs should account for CO2-specific issues related to propagating fractures for both brittle and ductile failure mechanisms.
- Understand the thermodynamics of the CO2 stream, including the effects of impurities, for the design and operation of the CO2 handling systems. Remember that depressurization can result in low temperatures (i.e., –78°C or –108°F) and solid CO2 formation.
- Thermal expansion can lead to system over-pressurization; appropriate system pressure relief should be included in the design.
- Liquid-phase CO2, and especially supercritical CO2, are highly efficient solvents. During a release, any impurities within the CO2 stream will be released — either in vapor with the CO2 plume or deposited in the vicinity of the release point. Addressing the potential hazards associated with the release of CO2 impurities is important.
As CCS projects are delivered and operational experience grows, the challenges associated with CO2 will become more familiar to engineers. The industry will mature with recognized codes, standards, and best practices.
In Closing
Handling large inventories of CO2 may be new to many involved with CCS implementation. For effective risk management, members of CCS projects must be aware of the properties and hazards associated with CO2. The CO2RISKMAN guidance includes the following key message (8, 9): “There is no reason why the major accident risks from a CO2 handling system within a carbon dioxide capture, transportation, and geological storage (CCS) operation cannot be low and well within acceptable limits. To achieve this will require the application of existing rigorous hazard management processes combined with an adequate understanding of the properties and behaviors of CO2.”
A longer version of this article is scheduled to appear in Process Safety Progress.
Literature Cited
- United Nations, “Globally Harmonized System of Classification and Labelling of Chemicals (GHS),” 8th revised edition, New York, NY and Geneva, Switzerland (2019).
- U.S. Dept. of Labor, “OSHA Occupational Chemical Database: Carbon Dioxide,” https://www.osha.gov/chemicaldata/183 (accessed April 2023).
- U.S. Centers for Disease Control and Prevention, “NIOSH Publications and Products. Immediately Dangerous to Life or Health Concentrations: Carbon Dioxide,” www.cdc.gov (accessed April 2023).
- U.S. Food Safety and Inspection Service, “Carbon Dioxide Health Hazard Information Sheet,” https://www.fsis.usda.gov/sites/default/files/media_file/2020-08/Carbon-Dioxide.pdf (2020).
- U.S. Dept. of Transportation, “Failure Investigation Report - Denbury Gulf Coast Pipelines, LLC – Pipeline Rupture/ Natural Force Damage,” www.phmsa.dot.gov/sites/phmsa.dot.gov/files/2022-05/Failure%20Investigation%20Report%20-%20Denbury%20Gulf%20Coast%20Pipeline.pdf (2022).
- U.S. Environmental Protection Agency, “Carbon Dioxide as a Fire Suppressant: Examining the Risks,” https://www.epa.gov/sites/default/files/2015-06/documents/co2report.pdf (Feb. 2000).
- Clayton, W. E., et al., “Catastrophic Failure of a Liquid Carbon Dioxide Storage Vessel,” Process Safety Progress, 13 (4), pp. 202–209 (1994).
- DNV, “CO2RISKMAN JIP: Guidance on CCS CO2 Safety and Environment Major Accident Hazard Risk Management. Level 2 – Overview,” Report No. I3IJLJW-2, Rev. 3 (2021).
- DNV, “CO2RISKMAN JIP: Guidance on CCS CO2 Safety and Environment Major Accident Hazard Risk Management. Level 3 – Generic Guidance,” Report No. I3IJLJW-2, Rev. 3 (2021).
- Metz, B., et al. (eds.), “IPCC Special Report on Carbon Dioxide Capture and Storage,” Prepared by Working Group III of the Intergovernmental Panel on Climate Change (IPCC), Cambridge University Press, Cambridge, U.K., and New York, NY (2005).
- Peletiri, S. P., et al., “Effects of Impurities on CO2 Pipeline Performance,” Chemical Engineering Transactions, 57, pp. 355–360 (2017).
- DNV, “Design and Operation of Carbon Dioxide Pipelines,” Report No. DNV-RP-F104 (2021).
- International Organization for Standardization, “Risk Management – Guidelines,” ISO 31000, Geneva, Switzerland (2018).
- Center for Chemical Process Safety, “Guidelines for Risk Based Process Safety,” CCPS, American Institute of Chemical Engineers, New York, NY (2007).
Copyright Permissions
Would you like to reuse content from CEP Magazine? It’s easy to request permission to reuse content. Simply click here to connect instantly to licensing services, where you can choose from a list of options regarding how you would like to reuse the desired content and complete the transaction.