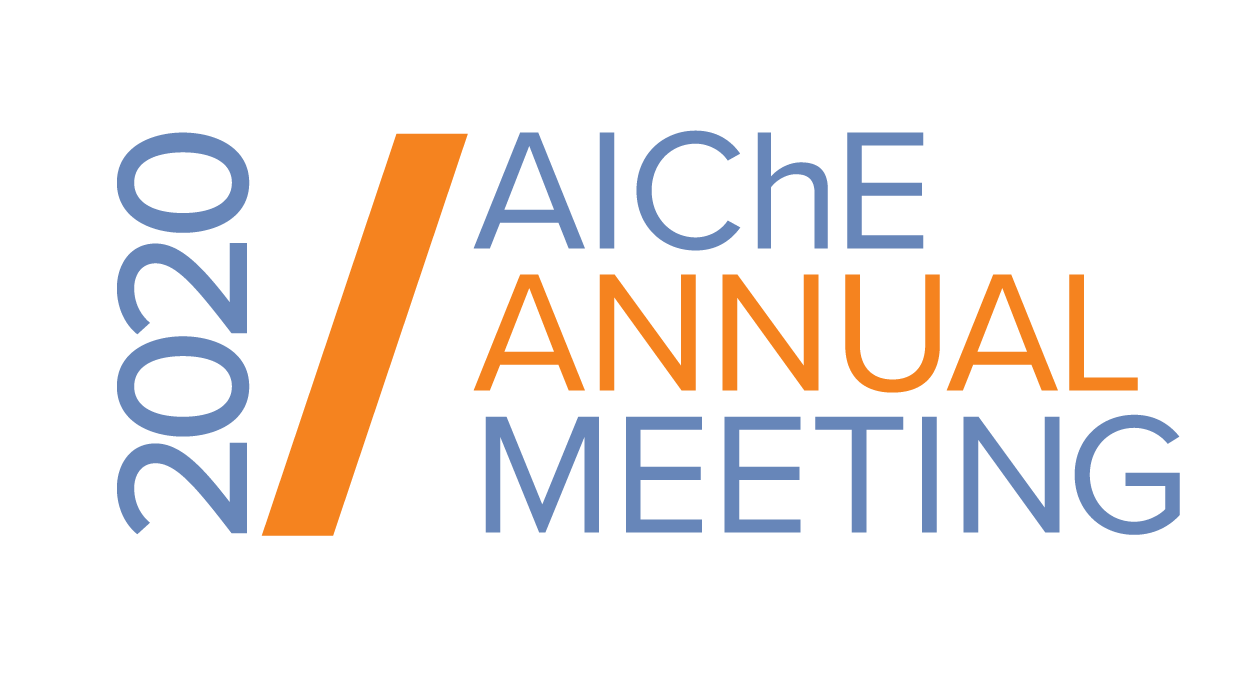
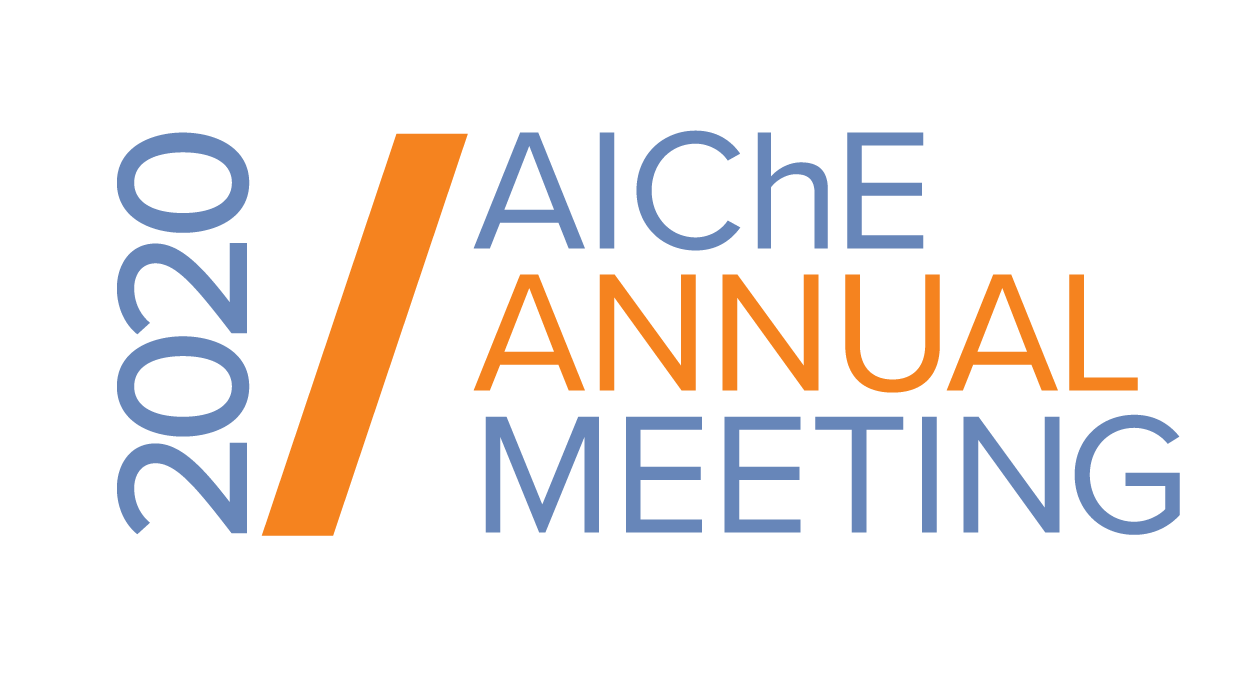
Setting the scene for solar fuels: decarbonizing aviation
Two targets are particularly prominent on the global agenda. The aviation industry has set a target of â50% reduction of CO2 emission in 2050 compared to 2005â (ATAG target) [2]. To translate the magnitude of the challenge into the metrics of the renewable fuel domain, we show that in 2050 a challenging 90% emission reduction and a production volume of renewable SPK >550 Mt/a is required to meet the ATAG target. The ratio of CO2 emissions in 2050 compared to 2005 can be represented as the product of (1) the growth in revenue passenger kilometers (RPK) according to estimates of the industry (red, approximately doubling every 15 years, or 4.7%/a growth rate), (2) the efficiency gains of aircraft and operations (green, assuming 1.5-2%/a efficiency gain) and (3) the fraction of substitution of fossil energy by âzero-carbon energyâ (blue) required to meet the ATAG target (Figure 1, taken from ref. 3). Obviously, the path towards deep emission reduction requires the use of alternative jet fuels. The five to six-fold increase in fuel burn (green) in 2050 at 50% of emissions relative to 2005 requires then to remove approximately 90% of the emissions with the use of alternative jet fuel of several hundred megatons per year globally and several tens of megatons per year in Europe [4].
The second target, set out by the Paris Agreement, concerns the limitation of global warming [5]. According to the IPCC 2018 Special Report (on the impacts of global warming of 1.5 °C above pre-industrial levels and related global greenhouse gas emission pathways), âall pathways that limit global warming to 1.5°C [...] project the use of carbon dioxide removal (CDR) [...] to compensate for residual emissions and, in most cases, achieve net negative emissions to return global warming to 1.5°C following a peakâ [6]. The feasibility and sustainability of CDR via direct air capture (DAC) of CO2 and storage is one option in a wider portfolio of technologies.
Technology pull for CO2-based solar fuel and direct air capture
On the one hand, the âtechnology pullâ of the two targets promotes the development of cost-effective DAC as a necessary resource of renewable CO2 at a scale of gigatonnes p.a. for the global demand of carbon-neutral renewable SPK for aviation to fulfil the ATAG target. On the other hand it promotes DAC as a technology option for the negative emission regime from 2050 on to limit global warming to 1.5 °C according to the IPCC report. The technology pull for DAC-CO2-based fuels is also motivated by the fact that current biofuel technology does not meet the sustainability and availability requirements at the scale of future global fuel demand [7]. A more efficient technology for storing solar energy with smaller CO2 footprint is the production of solar thermochemical fuels from water and non-fossil CO2.
The solar thermochemical fuel pathway
CO2 and H2O are converted into a mixture of CO and H2 (syngas) through a redox cycle of a metal oxide operated in a temperature-pressure swing using concentrated solar energy as the source of heat [8,9]. The produced syngas is then converted into liquid hydrocarbons in a Fischer-Tropsch synthesis (FT).
The energy conversion efficiency of the solar thermochemical step is the most relevant performance indicator and the key to economic competitiveness. This is because â analogous to concentrated solar power (CSP) plants, where the solar concentrating subsystem represents about half of the total investment cost â higher efficiency implies a smaller solar concentrating system for the same fuel yield, which results in lower specific fuel cost. The long-term goal is 20% to ensure commercial viability [10].
Research on the solar thermochemical fuel pathway has progressed significantly over the last years, e.g. in the European projects SOLAR-JET and SUN-to-LIQUID, progressing the energy conversion efficiency from <1% to ca. 1.7% [8,11] and further to 5.6%±1% [12], respectively. In the future, the novel ordered redox materials [13] (enabling homogeneous reactor temperatures and higher mass loadings, increasing the yield of the process) and the recuperation of heat from the thermal redox cycle [14,15] represent an opportunity to significantly enhance the efficiency.
Life-cycle emission reduction potential
For a baseline case with CO2 from DAC and a thermochemical reactor efficiency of 19%, the greenhouse gas emissions of the solar thermochemical fuel pathway are shown in Figure 2 (taken from ref. 16). As can be seen, the favorable environmental performance is achieved through counterbalancing the positive emissions by negative emissions through air capture of CO2.
Geographical techno-economic potential
Through the exclusion of unsuitable areas, the net available land for solar fuel production is identified. The following areas are excluded: areas with existing ground structures, water bodies, shifting sands, slopes â¥5%, protected areas, as well as areas covered by forest, closed shrubland, woody savannas, wetland, cropland, urban settlements, or snow/ice. In the United States, 0.6Ã10^6 km^2 or about 8% of the contiguous country area is available, mostly in the Southwest. Here, the USA has highly irradiated areas and very favorable costs of capital, enabling lowâcost production of solar fuels (Figure 3, left; green line indicates US jet fuel demand and the red line (in both graphs) the global demand. Figure taken from ref. 17). In the baseline case with an assumed reactor efficiency of 19%, production costs of under 1.75 â¬/L can be achieved, whereas the national demand for jet fuel could be covered at costs between 1.74 and 1.82 â¬/L, and at average costs of 1.79 â¬/L (global demand: 1.74-1.88 â¬/L; average 1.84 â¬/L). For a reactor efficiency of 25%, production costs of under 1.6 â¬/L are possible at the best locations.
Assuming a global perspective, the production costs can be further decreased in very sunny locations with favorable financial conditions such as Chile. The right diagram in Figure 3 shows the costâsupply curves for the baseline case with varying nominal weighted average cost of capital (WACC) between countries, and constant nominal WACC in each country of 4% and 10%, respectively. The colors of the pie charts indicate the distribution of total production volumes over the regions for each case in the different regions of Europe, North Africa, and the Middle East (MED, red), the USA (USA, blue), and South America (SAM, green) (Figure taken from ref. 17).
Roadmap for development of the thermochemical reactor efficiency
The main technology gap and the main risks for the further development of the fuel path indisputably relate to the thermochemical solar reactor subsystem. The main drivers of efficiency improvement are seen to be the adoption of ordered structures for the redox material that will enable a homogeneous temperature distribution in the reactor and heat recuperation. Proposed maturity gates for the solar thermochemical reactor efficiency on the way to commercialization are 10-15% in 2020-2025, 15-20% in 2025-2030, and >20% from 2030 on.
[1] https://eur-lex.europa.eu/legal-content/EN/TXT/HTML/?uri=CELEX:52019DC0640&from=EN
[2] www.atag.org
[3] A. Sizmann, 4th Bauhaus Luftfahrt Symposium, 2019
[4] Transport and Environment, Roadmap to decarbonising European aviation, 2018, https://www.transportenvironment.org/sites/te/files/publications/2018_10_Aviation_decarbonisation_paper_final.pdf
[5] 21st Conference of Parties of the UNFCCC to adopt the Paris Agreement, Decision 1/CP.21, par. 21, 2016
[6] IPCC: Summary for Policymakers. In: Global warming of 1.5°C. An IPCC Special Report [V. Masson-Delmotte, et al. (eds.)]. World Meteorological Organization, Geneva, Switzerland, 2018
[7] A. Mohr, S. Raman, Energy Policy, 63, 2013, 114; S. Y. Searle, C.J. Malins, Proceedings of the 20th European Biomass Conference and Exhibition, Milan, 53, 2012, and references therein.
[8] W.C. Chueh, C. Falter, et al., Science, 330, 2010, 1797.
[9] M. Romero, A. Steinfeld, Energy Environ. Sci. 5, 2012, 9234.
[10] E.B. Stechel, J.E. Miller, J. of CO2 Utilization, 1, 2013, 28.
[11] D. Marxer, P. Furler, J. Scheffe, et al., Energy Fuels, 5, 2015, 3241
[12] S. Zoller, Diss. ETH No. 26451, 2020
[13] M. Hoes, S. Ackermann, D. Theiler, et al., Energy Technology, 2019, 1900484
[14] J. Scheffe, A. Steinfeld, Energy & Fuels, 26, 2012, 1928
[15] C. Falter, R. Pitz-Paal, Solar Energy, 2018, 176, 320
[16] C. Falter, A. Valente, A. Habersetzer, et al., SUN-to-LIQUID (654408) Public Report D1.7, 2019.
[17] C. Falter, N. Scharfenberg, A. Habersetzer, Energies, 13, 2020, 802